Genetic and epigenetic alterations in pancreatic neuroendocrine tumors
Introduction
Neuroendocrine tumors (NETs) are a heterogenous group of tumors that originate from neuroendocrine cells, mainly in the gastrointestinal and bronchopulmonary tracts (1). The management of NETs consists of surgical resection, which is the only curative treatment, and medical, radionuclide, and/or interventional liver-directed therapy for patients with unresectable NET metastases. NETs are subclassified into three grades, G1, G2, and G3, based on two histologically derived proliferative indices: the rate of mitoses per 10 high-power fields and the percentage of cells positively stained for the nuclear antigen Ki-67 (2). There have been significant discoveries of the underlying genetic and epigenetic events involved in the initiation and progression of low-grade NETs, both in small intestine (3) and pancreatic NETs (PNETs) (4).
Although NETs are considered rare, the incidence of NETs has increased markedly in recent decades, reaching an annual incidence of 6.98 cases per 100,000 individuals (1,5), with 0.33 cases per 100,000 individuals for PNETs, accounting for 3% of all pancreatic tumors (6) and 15–20% of all gastro-entero-pancreatic (GEP) NETs (7). Most PNETs (60–85%) are nonfunctioning (not causing a clinical syndrome secondary to hormonal oversecretion). The clinical syndromes caused by functional PNETs depend on the cell from which the PNET originates; insulinoma from beta cells (resulting in hypoglycemia), gastrinoma from G-cells (resulting in gastric ulcers, called Zollinger-Ellison syndrome), vasoactive intestinal peptide (VIP) (resulting in watery diarrhea syndrome), and glucagonoma from alpha-cells (causing diabetes and skin erosions) (8-10).
The natural history of nonfunctioning and functioning PNETs is distinct, both in terms of the primary lesion(s) size, location, and multifocality and in terms of the risk for metastatic disease. In addition, different PNET types have a higher risk of association with hereditary syndromes, such as the association of gastrinoma and insulinoma with multiple endocrine neoplasia type 1 (MEN-1) syndrome. The different biological behavior is tightly associated with the genetic and epigenetic characteristics of the different tumor types, as will be discussed in this review.
The clinical syndromes associated with functional PNETs lead to earlier diagnosis of PNETs compared to nonfunctioning PNETs. The latter may be detected when a neighboring structure is compressed/involved by the tumor (e.g., obstruction of the pancreatic/bile duct) or, incidentally, when a CT/MRI scan is performed for a different indication (8). The lack of tumor functionality may lead to a more advanced stage of the tumor at diagnosis since most patients will be asymptomatic; however, genetic and epigenetic factors may also contribute to this risk, as will be discussed in this review.
Of all PNETs, 7% have a KI67 >20% (G3 NET), and even a small subset of those are poorly differentiated (PD) neuroendocrine carcinomas (NECs) (11). The natural history of these tumors and the mortality risk are different. In addition, the diagnostic tools for the two groups are different, especially in terms of the selection of functional imaging modality; 18F-FDG PET/CT is superior to somatostatin receptor (SSTR)-based PET imaging in PD NECs due to the high metabolic activity of the tumor cells and the lower SSTR expression levels (12). Furthermore, intervention choices are different since patients harboring PD NECs are treated with chemotherapy while those with G3 PNETs can be treated with surgery, somatostatin analogues (13-15) and/or other targeted agents (everolimus, sunitinib) (16,17), and peptide receptor radionuclide therapy (177Lu-DOTATATE) (18). The genetic landscape of these tumors is also distinct, with different altered signaling pathways, mutation rates, and, consequently, different tumor behaviors.
While most PNETs are sporadic, about 10–20% of all PNETs occur as part of inherited syndromes (19,20). The main syndromes associated with increased risk for developing PNETs include MEN1 due to germline mutations in the MEN1 gene, von Hippel-Lindau disease (VHL) due to germline mutations in the VHL gene, tuberous sclerosis complex (TSC) due to germline mutations in the TSC1 or TSC2 genes, neurofibromatosis type 1 due to germline mutations in the NF1 gene, and Cowden syndrome due to germline mutations in the PTEN gene. Analysis of VHL- and MEN1-related PNETs, and of sporadic PNETs with relatively high rates of somatic MEN1 gene mutations, has provided an understanding of the genetic and epigenetic mechanisms leading to PNET development.
Genetics
The genetic background of PNETs is strongly associated with the differentiation level/grade, the functional status of the tumor, and the aggressiveness of the tumor. In the following section, we describe the genetic changes associated with PNET subgroups.
Well differentiated
Nonfunctioning pancreatic NETs
A study by Missiaglia and colleagues described the differential expression of several genes associated with the AKT/PI3K/mTOR pathway in PNETs (21). In this study, a distinct transcriptomic signature for G1 PNET, PD-PNET, insulinomas, and normal islet cells was reported using genome-wide gene expression analysis. In addition, the investigators validated, using quantitative PCR, the differential expression of TSC2 and PTEN, both encoding proteins in the PI3K/AKT/mTOR pathways. Furthermore, high protein expression was associated with better progression-free survival (TSC2, PTEN) and overall survival (TSC2) (21).
In 2011, Jiao and associates performed whole-exome sequencing in 10 PNETs, with targeted sequencing in an additional 58 PNET samples (22). The investigators reported a high rate (44%) of somatic mutations in MEN1 and in genes involved in the mTOR pathway [6 in TSC2, 5 in PTEN, and 1 in PI3CA in the entire study cohort (N=68)]. In this study, 43% of the samples had mutually exclusive damaging mutations in two chromatin remodeling complex genes, DAXX and ATRX. The proteins encoded by ATRX and DAXX are required for incorporating the histone variant 3.3 to chromosome telomeres. In the same year, Heaphy and colleagues reported a follow-up study (23) that compared the presence of alternative lengthening of telomeres (ALT) in PNETs by their ATRX/DAXX mutation status. In this study, all 19 tumor samples with ATRX/DAXX mutations had abnormal ALT by fluorescent in situ hybridization (FISH) techniques. In tumors with wild-type ATRX/DAXX sequences, only 6 of 20 had a similar pattern, but all had abnormal nuclear expression of ATRX or DAXX, whereas all 14 remaining tumors had normal DAXX and ATRX expression.
A comprehensive genomic analysis of PNETs using whole-genome sequencing was published by Scarpa and colleagues (4). The analysis included 98 samples (4) that were processed for germline and somatic whole-genome sequencing and for telomere length. In addition to the validation of a high DAXX/ATRX mutation rate (33 of 98 cases) and the presence of ALT, the researchers were able to further narrow the “dark matter” (samples without known driver gene mutations): in samples with wild-type ATRX/DAXX and no ALT, a high rate of gene rearrangements and novel fusion-genes were identified, including the Ewing sarcoma 1 (EWSR1) gene. The germline DNA analysis was also informative, demonstrating for the first time repeating pathogenic variants in MUTYH, a known cause of hereditary colon polyposis (5 cases) (24), and CHEK2, associated with an increased risk of breast and ovarian cancer (4 cases) (25,26). Additional germline pathogenic variants were detected in BRCA2 and CDKN1B (1 case each) and in genes known to be associated with increased risk for PNET, MEN1, and VHL (5 and 1 cases, respectively). The rate of germline DNA pathogenic variants was 17%, emphasizing the need for a low-suspicion threshold for hereditary syndromes in patients who have PNETs. Analysis of the somatic variants and copy number (CN) variant analysis in the 98 cases defined 4 main driver molecular pathways for PNET initiation and/or progression: DNA damage repair (MUTYH, CHEK2, BRCA2), chromatin modifiers (SETD2, MLL3), ALT (ATRX, DAXX), and the mTOR signaling pathway (TSC1, TSC2, PTEN, and DEPDC5), with MEN1 having a role in each of these pathways. In addition to four previously implicated pathways, a new pathway was described and named MUTYH after the gene that was mutated in the germline DNA of most of the cases with this gene signature. PNETs with a dominant MUTYH signature had the highest mutation burden. Interestingly, in the study by Jiao and colleagues, PNETs with ATRX or DAXX mutations were associated with a better prognosis (22), while several other studies indicated poor prognosis in patients harboring these mutations, suggesting that DAXX/ATRX mutations are either a cause or consequence of disease progression (4,27-29). Moreover, tumors with altered ATRX/DAXX are more susceptible to the development of chromosomal alterations (28). The impacts of DAXX/ATRX/MEN1 mutations on tumor biology and methylomes are detailed later in this review in the Epigenetics section.
CN variations are of high interest in NETs, especially considering the high rate of chromosome 18 CN loss in small intestine NETs (SINETs) (30) and its association with the clinical outcome (3). In line with the distinct genomic landscape of PNETs versus SINETs, CN variants in nonfunctional PNETs include CN losses of 11q (including the MEN1 encoding locus at 11q13); CN gains in chromosomes 11p, 10q, 6q, 3p, 1p, and 1q; and gains in 17q, 7q, 20q, 9p, 7p, and 9q (31). Among these, CN losses of chromosomes 3, 6q (32), and 1 (33) and A CN gain of chromosome 17q (32) have been associated with increased risk of liver metastases.
Functioning PNETs
Functioning PNETs have distinct genetic characteristics compared to nonfunctioning tumors. Furthermore, even within the group of insulin-secreting PNETs, those localized to the pancreas differ from metastatic insulinoma genetically and in other molecular features, such as expression of SSTR, which is more commonly expressed in metastatic insulinomas (12).
Insulinoma
In insulin-secreting PNETs, the gene with the highest rate of somatic mutation is the Yin Yang 1 (YY1) gene. In the Caucasian (German) population (34), the mutation rate was 13%, with all the mutations detected in women with sporadic insulinomas, and all were the Thr372Arg missense variant. In the Asian population, however, the rate of YY1 pathogenic variants in sporadic insulinoma was 30% (35).
The differences in the rates of CN variations between nonfunctioning PNETs and insulinomas are striking. Benign insulinoma have lower rates of any CN variation (losses/gains) compared to nonfunctioning PNETs and very low rates of 6q, 3p, and 10q CN losses and 17q and 9p CN gains (all <10%). In contrast, malignant insulinomas have 6q CN losses in 70% of tumor samples and CN gains in 17q, 7q, 20q, and 7p (frequency of 57%, 47%, 43%, and 37%, respectively), all higher than either nonfunctioning PNETs or benign insulinomas. This demonstrated the distinct nature of nonfunctioning PNETs, benign insulinomas, and malignant insulinomas (31).
Gastrinoma
Gastrinoma is associated with MEN1 syndrome in 25% of patients; hence, whether duodenal or pancreatic, this diagnosis should drive the clinician to screen for additional manifestations (primary hyperparathyroidism, pituitary adenoma) and to perform genetic testing for germline MEN1 mutations (36). In contrast to the unique genetic patterns in insulinomas, sporadic gastrinomas are characterized by a high rate of MEN1 somatic mutations (37). In one study, 31% of 51 sporadic gastrinomas had an MEN1 somatic mutation, with clustering of 9 of 16 mutations detected in exon 2, in contrast to the non-specific distribution in germline MEN1 mutations (38). An even higher rate of MEN1 mutations, 7 of 12 tumors (58%), was reported in a small study of sporadic gastrinomas in Japanese patients (39).
Rates of CN variations are very low in gastrinomas compared to nonfunctioning PNETs and insulinomas. Deletions in 1q (40) were detected in 12 of 27 gastrinomas (44%) and were associated with more aggressive disease. In a small cohort of PNETs (41), CN loss of 22q loss was identified in 8 gastrinomas and in additional PNETs. CN gain in 9p, including the JAK2 locus, was detected in 29% of sporadic gastrinomas (31). A study with a small sample size identified somatic variants in the CDKN2A gene (42). In contrast, another study did not observe any mutation or loss of heterozygosity (LOH) in the 9p locus. However, promoter hypermethylation has been reported in the CDKN2A gene in 52% (25 of 44) of tumors (43).
High-grade and poorly differentiated pancreatic neuroendocrine carcinomas
G3 NETs and NECs are highly aggressive neoplasms, which are typically non-operable on presentation due to the presence of miliary metastatic disease and rapid recurrence or disease dissemination after surgical resection. Hence, systemic treatment with chemotherapy is usually indicated (11). G3 NETs are very heterogenous, as reflected by the wide range of the proliferative index Ki-67 (>20–100%) defining these tumors. Hence, there is ongoing research on the optimal subclassification of this group, with several features used for subclassification of these tumors. Morphologically, well-differentiated cells define G3 NETs, while PD cells define NECs, which are further subdivided into small- and large-cell NECs. The proliferation index, Ki-67, has been also suggested for subclassifying G3 NETs (44), with Ki-67 <55% used as the cut-off based on its association with a lower risk of mortality (44). In addition, high rates of abnormal immunohistochemical staining for protein products of the tumor suppressor genes TP53 and RB1 were reported in pancreatic NECs, with correlation to genetic alterations in the corresponding genes (45). In contrast to the advances in G1/G2 NET, genetic studies and data on G3 NENs are scant.
In contrast to the distinct genetic landscape between well-differentiated NET of the pancreas, as detailed above, and of the small intestine NETs, with repeating variant alterations in the CDKN1B gene and chromosomal alterations in chromosomes 18, 4, and 5 (3,30,46), the genetic alterations in PD NECs is similar across different anatomic locations. PD NECs are characterized by loss of the retinoblastoma tumor suppressor gene (RB1) and/or by aberrant expression of TP53 and corresponding expression of the encoded protein, as demonstrated by the suggestion that immunohistochemistry is a distinctive tool that can be used to differentiate between WD and PD NETs (47,48).
Actionable mutations in PNETs
The ultimate goal of personalized medicine is to identify a targetable alteration in a specific patient that would allow for matching a highly effective and safe treatment to each patient. Actionable mutations are DNA alterations that are known to be associated with a response to a specific therapy that target them. In WD-PNETs, the most attractive pathway is mTOR since there is an FDA approved intervention, everolimus, which is also found to be effective in patients with PNETs, both in terms of decreasing disease progression and improving overall survival (17,49). To determine if such a strategy can be used in patients with unresectable PNETs, a phase II mutation-targeted therapy study was conducted. In the study, tumor tissues (eight PNETs) were sequenced using a oncogene and tumor suppressor gene panel (50), and treatment with everolimus was indicated in the presence of gene alterations involved in the mTOR-pathway and with sunitinib for all other mutations or wild type tumors. The researchers reported a very low number of driver mutations and variants in general, while a high rate of CN alterations was detected when no driver mutations were found (46). A recent study reported BRAF pathogenic mutations in 8% of WD-PNETs, including both V600E and non-V600E variants (among those, the only recurrent mutation was K601E) (51). The authors showed a gradual decrease in cell viability using the RAF inhibitor, dabrafenib, which was most potent in the V600E variants and the K601E variants. Furthermore, a combination of dabrafenib with the MEK inhibitor, trametinib, induced a decrease in cell viability in cells with the V600E and K601E BRAF mutation, but not in wild-type BRAF PNET cells. This finding, however, has not been translated to the clinic to test the efficacy of using a BRAF inhibitor alone or in combination with a MEK inhibitor in patients with unresectable PNETs that are BRAF mutants.
Epigenetics
Epigenetics refers to a molecular mechanism by which gene expression is regulated without a direct change to the gene sequence. These mechanisms include CpG methylation, histone modification, and non-coding RNA molecules (long, micro). In the current review, we focus on DNA methylation status in PNETs since they have been more broadly studied. The amount of dark molecular matter in PNETs, even when whole-genome sequencing is performed, and the high rate of somatic mutations in MEN1, which is known to affect DNA methylation, suggest a significant role of epigenetics in the development and progression of PNETs.
DNA methylation
DNA methylation regulates gene expression through promoter/enhancer methylation. In cancer cells, dysregulated CpG methylation is characterized by downregulated gene expression due to promoter hypermethylation, resulting in silencing of tumor suppression genes and tumor development. In addition, another hallmark of cancer is genome-wide hypomethylation, which may lead to DNA instability and an increased risk of structural changes. DNA methylation is a dynamic process that is regulated by methylating and demethylating enzymes. DNA n-methyl transferases (DNMTs) add a methyl group to the cytosine in CpG DNA sequences, and demethylating enzymes remove the methyl group and include enzymes from the ten-eleven translocation (TET) enzyme family.
Analysis of DNA methylation (methylome) are completing a transition similar to the one witnessed for the advances in DNA sequencing with the development of high-throughput techniques. Currently, the use of arrays, such as the Illumina Infinium arrays, enables quantification of DNA methylation levels in up to ~850,000 CpG sites, including coding and non-coding regions (52).
Whole-genome DNA methylation analysis of 29 PNETs revealed hypermethylation in MEN1-associated PNETs compared to sporadic and VHL-associated PNETs (53). The analysis, integrated with gene expression data, demonstrated a significantly higher number of hypermethylated CpG in the MEN1 group and a higher number of genes with both hypermethylation and downregulated expression. Moreover, CpG island methylator phenotype (CIMP) analysis revealed promoter hypermethylation and gene downregulation in three genes: SFRP5 in sporadic PNETs and CDCA7L and RBM47 in MEN1-related PNETs. A fascinating study by Chang and associates demonstrated that PNETs with ATRX, DAXX, or MEN1 mutations share common methylation signatures, distinct from tumors without mutations in these genes (29). Moreover, the ATRX/DAXX/MEN1-aberrant PNET methylation signature is similar to the alpha-cell methylation pattern, including upregulation of the characteristic HNF1A and its target genes. The mechanism suggested by the authors is trans-differentiation of the tumor cells during their evolvement. This finding was further supported by a study by Cejas and colleagues (54).
In the targeted CpG promoter methylation analysis of PNETs (n=46), hypermethylation in several genes, which were selected due to their frequent mutation in NETs or their epigenetic regulation and involvement in other tumor types, was identified. The genes with a high rate of promoter hypermethylation were MEN1 (19% of tumors) and MGMT (methylguanine-O6-methyl transferase) (17% of tumors), a gene encoding DNA de-alkylating enzyme that may affect the response to treatment with the alkylating agent temozolomide (55), and it is currently being investigated as a therapy for NETs (11,56-58). APC (adenomatous polyposis coli), responsible for APC syndrome and the syndrome of gastric adenocarcinoma and proximal polyposis of the stomach (GAPPS) (59,60), had promoter hypermethylation in 48% of the tumors and had reduced expression in PNETs. Interestingly, the APC gene is involved and regulated by the Wnt pathway, which is not one of the PNET pathways with gene mutations (61). Additional genes that had promoter hypermethylation in these samples were hypermethylated in cancer 1 (HIC1), associated with the TP53 tumor suppressor gene, which was found to be altered in 93% of the samples, and Ras association domain family 1 isoform A (RASSF1A), which may regulate apoptosis, mitotic, and cell-cycle arrest through various pathways (62). Hypermethylation in the CDKN2A gene, another cell cycle modulating gene, has also been reported in PNETs, with a high rate of hypermethylation in gastrinomas (43).
Genetic predisposition to PNET syndromes
The risk for hereditary syndromes is relatively high among patients with PNETs, with 17% of patients with apparently sporadic PNETs having a germline mutation (4). Cardinal clinical and pathologic features for hereditary/genetic predisposition for PNETs include young age at diagnosis, tumor multifocality, and positive family history of PNETs (or other relevant neoplasms), functioning tumors, and metastatic disease. Manifestations associated with a specific syndrome should raise suspicion and lead to genetic testing and counseling. For example, the presence of a history of renal cell carcinoma and/or hemangioblastoma, pancreatic cysts, and PNETs in a patient suggest a diagnosis of vHL, gastrinomas are part of MEN1 in 10% of cases, and diagnosis with primary hyperparathyroidism, kidney stones, and/or pituitary tumors in a patient suggests MEN1 syndrome (36).
MEN1
MEN1 syndrome is inherited in an autosomal dominant fashion. About 80% of patients with a known disease-causing MEN1 mutation will develop clinical manifestations of the disease. The overall rate of PNETs in MEN1 patients ranges between 30% and 80%. The most distinct characteristic of MEN1-related PNETs is the high rate of gastrinomas (40%), which constitute >50% of all pancreaticoduodenal NETs in patients with MEN1. However, gastrinomas are usually non-pancreatic in origin. A diagnosis of gastrinoma is associated with a 20% risk of being diagnosed with MEN1 syndrome. Additional tumor types in patients with MEN1-related PNETs include nonfunctioning PNETs in up to 55% of patients, insulinoma in 10% of patients, and, rarely, VIP- and glucagon-secreting PNETs (<1% each).
MEN1-related neoplasms are characterized by genome-wide hypermethylation, at least in part due to increased expression of the DNA methylating enzyme DNMT1 (63). MEN1-related PNETs are also characterized by genome-wide hypermethylation (53) and by a high rate of promoter hypermethylation, as recently shown in a targeted methylation analysis (64). These findings may explain the lack of consistent genotype–phenotype associations in MEN1.
VHL
VHL disease is inherited as an autosomal-dominant trait, with an incidence of 1 case per 36,000 live births and with high (>90%) penetrance. The gene responsible for the disease is VHL (OMIM 193300), located in chromosome 3 (3q22), encoding the VHL protein (65). The VHL protein functions as the recognition unit for HIF1a in the ubiquitin system, and its malfunction leads to high HIF1a cellular levels and pseudohypoxia (66), with the development of multiple hyper-vascular lesions, including neoplasms and cysts in the kidneys, catecholamines secreting adrenal medulla tumors (pheochromocytomas), hemangioblastomas of the brain and the retina, PNETs, and cysts and additional lesions (67,68). The risk of developing PNETs in patients with VHL ranges between 9–17%, with a 30–50% risk of multiple lesions (68,69).
In VHL syndrome, there is a genotype–phenotype association (Table 1). This was first reported for VHL-related pheochromocytomas that were rarely detected in patients without missense variants (72,73). In terms of risk for developing PNETs, several prospective and large cohort studies have been reported in the recent years, which analyzed such associations. Patients with a germline missense VHL mutation and/or a mutation within exon 3 of the VHL gene (74) have a higher risk of developing PNETs and a higher risk of developing metastatic disease during follow-up (70,75), compared to other mutation types and locations. Moreover, among patients with missense variant in the VHL gene, those with 167 codon variants of the VHL protein have an even higher risk of a poor clinical outcome (75,76). VHL-related lesions are characterized by various epigenetic alterations. Among them are global DNA hypomethylation, compared to normal islet cells, and sporadic and MEN1-associated PNETs (53). This may be part of the pseudohypoxic state, induced by unregulated HIF1α activity in these tumors.
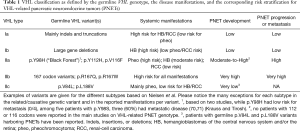
Full table
Hereditary syndromes with low PNET penetrance
Several additional hereditary genetic predisposition syndromes are associated with an increased risk of developing PNETs. Cowden syndrome is associated with a high risk of thyroid, breast, and endometrial carcinomas due to germline pathogenic mutations in the PTEN gene. As part of the PI3K/mTOR pathway, PTEN is a known driver of PNET tumorigenesis, and, in fact, the low rate of diagnosis with PNETs in patients with Cowden is somewhat surprising (77).
TSC is associated with skin lesions, central nervous system malformations with secondary seizures, and hamartomas/cell overgrowth in the heart, brain, and kidneys (78). Several case reports have described patients with germline pathogenic variants in TSC1 (79) and TSC2 (80), the genes causing the syndrome. In fact, the TSC2 germline variant was reported in a patient with PNETs and with no additional manifestations of TSC (80). Furthermore, mTOR inhibition was found efficacious for treating patients with a metastatic PNET with germline TSC2 mutations (81).
Summary
There has been pronounced progress in our understanding of the genetic and epigenetic alterations associated with PNETs. These include (I) the identification of driver gene mutations involved in four pathways involved in PNETs, (II) the poor prognostic implications of ALT and its association with ATRX/DAXX mutations, and (III) the recent findings of alpha cell signature in PNETs, specifically recognized by ARX/PDX1 enhancer status, enabling early accurate recognition of high-risk non-functional PNETs. Also, the MEN1-associated PNETs that have been characterized by genome-wide hypermethylation explain the increasing importance of DNA methylation in PNET tumorigenesis, especially considering the high rate of somatic MEN1 somatic mutations. However, there are several knowledge gaps that still need to be addressed, which may have translational potential to the clinical management of patients with PNETs. These include the tumorigenic mechanisms of hereditary syndromes related to PNETs, such as VHL, the implication of genetic/epigenetic aberrations on the immune landscape of PNETs and on the utility of immunomodulators in PNETs, and the identification of truly actionable molecular alterations in PNETs. Another area is the development of liquid biopsy for PNETs, which not progressed to a clinically useful level, and needs to be further evaluated, either through peripheral blood transcriptome, circulating tumor DNA mutations, and/or methylome signatures that mirror the natural history of PNETs.
Acknowledgments
Funding: None.
Footnote
Provenance and Peer Review: This article was commissioned by the Guest Editors (Callisia N. Clarke, Douglas B. Evans) for the series “Pancreatic Neuroendocrine Tumors” published in Journal of Gastrointestinal Oncology. The article was sent for external peer review organized by the Guest Editors and the editorial office.
Conflicts of Interest: Both authors have completed the ICMJE uniform disclosure form (available at http://dx.doi.org/10.21037/jgo.2020.03.11). The series “Pancreatic Neuroendocrine Tumors” was commissioned by the editorial office without any funding or sponsorship. The authors have no other conflicts of interest to declare.
Ethical Statement: The authors are accountable for all aspects of the work in ensuring that questions related to the accuracy or integrity of any part of the work are appropriately investigated and resolved.
Open Access Statement: This is an Open Access article distributed in accordance with the Creative Commons Attribution-NonCommercial-NoDerivs 4.0 International License (CC BY-NC-ND 4.0), which permits the non-commercial replication and distribution of the article with the strict proviso that no changes or edits are made and the original work is properly cited (including links to both the formal publication through the relevant DOI and the license). See: https://creativecommons.org/licenses/by-nc-nd/4.0/.
References
- Dasari A, Shen C, Halperin D, et al. Trends in the incidence, prevalence, and survival outcomes in patients with neuroendocrine tumors in the United States. JAMA Oncol 2017;3:1335-42. [Crossref] [PubMed]
- Perren A, Couvelard A, Scoazec JY, et al. ENETS consensus guidelines for the standards of care in neuroendocrine tumors: pathology: diagnosis and prognostic stratification. Neuroendocrinology 2017;105:196-200. [Crossref] [PubMed]
- Karpathakis A, Dibra H, Pipinikas C, et al. Prognostic impact of novel molecular subtypes of small intestinal neuroendocrine tumor. Clin Cancer Res 2016;22:250-8. [Crossref] [PubMed]
- Scarpa A, Chang DK, Nones K, et al. Whole-genome landscape of pancreatic neuroendocrine tumours. Nature 2017;543:65-71. [Crossref] [PubMed]
- Hallet J, Law CHL, Cukier M, et al. Exploring the rising incidence of neuroendocrine tumors: a population-based analysis of epidemiology, metastatic presentation, and outcomes. Cancer 2015;121:589-97. [Crossref] [PubMed]
- Lee MR, Harris C, Baeg KJ, et al. Incidence trends of gastroenteropancreatic neuroendocrine tumors in the United States. Clin Gastroenterol Hepatol 2019;17:2212-2217.e1. [Crossref] [PubMed]
- Fraenkel M, Kim M, Faggiano A, et al. Incidence of gastroenteropancreatic neuroendocrine tumours: a systematic review of the literature. Endocr Relat Cancer 2014;21:R153-63. [Crossref] [PubMed]
- Klöppel G. Classification and pathology of gastroenteropancreatic neuroendocrine neoplasms. Endocr Relat Cancer 2011;18:S1-16. [Crossref] [PubMed]
- Franko J, Feng W, Yip L, et al. Non-functional neuroendocrine carcinoma of the pancreas: incidence, tumor biology, and outcomes in 2,158 patients. J Gastrointest Surg 2010;14:541-8. [Crossref] [PubMed]
- Scardoni M, Vittoria E, Volante M, et al. Mixed adenoneuroendocrine carcinomas of the gastrointestinal tract: targeted next-generation sequencing suggests a monoclonal origin of the two components. Neuroendocrinology 2014;100:310-6. [Crossref] [PubMed]
- Garcia-Carbonero R, Sorbye H, Baudin E, et al. ENETS consensus guidelines for high-grade gastroenteropancreatic neuroendocrine tumors and neuroendocrine carcinomas. Neuroendocrinology 2016;103:186-94. [Crossref] [PubMed]
- Tirosh A, Kebebew E. The utility of 68Ga-DOTATATE positron-emission tomography/computed tomography in the diagnosis, management, follow-up and prognosis of neuroendocrine tumors. Future Oncol 2018;14:111-22. [Crossref] [PubMed]
- Rinke A, Wittenberg M, Schade-Brittinger C, et al. Placebo-controlled, double-blind, prospective, randomized study on the effect of octreotide LAR in the control of tumor growth in patients with metastatic neuroendocrine midgut tumors (PROMID): results of long-term survival. Neuroendocrinology 2017;104:26-32. [Crossref] [PubMed]
- Rinke A, Müller HH, Schade-Brittinger C, et al. Placebo-controlled, double-blind, prospective, randomized study on the effect of octreotide LAR in the control of tumor growth in patients with metastatic neuroendocrine midgut tumors: a report from the PROMID study group. J Clin Oncol 2009;27:4656-63. [Crossref] [PubMed]
- Caplin ME, Pavel M, Ćwikła JB, et al. Lanreotide in metastatic enteropancreatic neuroendocrine tumors. N Engl J Med 2014;371:224-33. [Crossref] [PubMed]
- Yao JC, Shah MH, Ito T, et al. Everolimus for advanced pancreatic neuroendocrine tumors. N Engl J Med 2011;364:514-23. [Crossref] [PubMed]
- Raymond E, Dahan L, Raoul JL, et al. Sunitinib malate for the treatment of pancreatic neuroendocrine tumors. N Engl J Med 2011;364:501-13. [Crossref] [PubMed]
- Strosberg J, El-Haddad G, Wolin E, et al. Phase 3 trial of 177 Lu-Dotatate for midgut neuroendocrine tumors. N Engl J Med 2017;376:125-35. [Crossref] [PubMed]
- Crona J, Skogseid B. GEP-NETS UPDATE: genetics of neuroendocrine tumors. Eur J Endocrinol 2016;174:R275-90. [Crossref] [PubMed]
- Mafficini A, Scarpa A. Genetics and epigenetics of gastroenteropancreatic neuroendocrine neoplasms. Endocr Rev 2019;40:506-36. [Crossref] [PubMed]
- Missiaglia E, Dalai I, Barbi S, et al. Pancreatic endocrine tumors: expression profiling evidences a role for AKT-mTOR pathway. J Clin Oncol 2010;28:245-55. [Crossref] [PubMed]
- Jiao Y, Shi C, Edil BH, et al. DAXX/ATRX, MEN1, and mTOR pathway genes are frequently altered in pancreatic neuroendocrine tumors. Science 2011;331:1199-203. [Crossref] [PubMed]
- Heaphy CM, de Wilde RF, Jiao Y, et al. Altered telomeres in tumors with ATRX and DAXX mutations. Science 2011;333:425. [Crossref] [PubMed]
- Mazzei F, Viel A, Bignami M. Role of MUTYH in human cancer. Mutat Res 2013;743-744:33-43. [Crossref] [PubMed]
- Eoh KJ, Kim JE, Park HS, et al. Detection of germline mutations in patients with epithelial ovarian cancer using multi-gene panels: beyond BRCA1/2. Cancer Res Treat 2018;50:917-25. [Crossref] [PubMed]
- Allinen M, Huusko P, Mäntyniemi S, et al. Mutation analysis of the CHK2gene in families with hereditary breast cancer. Br J Cancer 2001;85:209-12. [Crossref] [PubMed]
- Singhi AD, Liu TC, Roncaioli JL, et al. Alternative lengthening of telomeres and loss of DAXX/ATRX expression predicts metastatic disease and poor survival in patients with pancreatic neuroendocrine tumors. Clin Cancer Res 2017;23:600-9. [Crossref] [PubMed]
- Marinoni I, Kurrer AS, Vassella E, et al. Loss of DAXX and ATRX are associated with chromosome instability and reduced survival of patients with pancreatic neuroendocrine tumors. Gastroenterology 2014;146:453-60.e5. [Crossref] [PubMed]
- Chan CS, Laddha SV, Lewis PW, et al. ATRX, DAXX or MEN1 mutant pancreatic neuroendocrine tumors are a distinct alpha-cell signature subgroup. Nat Commun 2018;9:4158. [Crossref] [PubMed]
- Francis JM, Kiezun A, Ramos AH, et al. Somatic mutation of CDKN1B in small intestine neuroendocrine tumors. Nat Genet 2013;45:1483-6. [Crossref] [PubMed]
- Capurso G, Festa S, Valente R, et al. Molecular pathology and genetics of pancreatic endocrine tumours. J Mol Endocrinol 2012;49:R37-50. [Crossref] [PubMed]
- Speel EJ, Scheidweiler AF, Zhao J, et al. Genetic evidence for early divergence of small functioning and nonfunctioning endocrine pancreatic tumors: gain of 9Q34 is an early event in insulinomas. Cancer Res 2001;61:5186-92. [PubMed]
- Ebrahimi SA, Wang EH, Wu A, et al. Deletion of chromosome 1 predicts prognosis in pancreatic endocrine tumors. Cancer Res 1999;59:311-5. [PubMed]
- Lichtenauer UD, Di Dalmazi G, Slater EP, et al. Frequency and clinical correlates of somatic Ying Yang 1 mutations in sporadic insulinomas. J Clin Endocrinol Metab 2015;100:E776-82. [Crossref] [PubMed]
- Cao Y, Gao Z, Li L, et al. Whole exome sequencing of insulinoma reveals recurrent T372R mutations in YY1. Nat Commun 2013;4:2810. [Crossref] [PubMed]
- Thakker RV, Newey PJ, Walls G V, et al. Clinical practice guidelines for multiple endocrine neoplasia type 1 (MEN1). J Clin Endocrinol Metab 2012;97:2990-3011. [Crossref] [PubMed]
- Pasieka JL, Chambers AJ. Gastrinoma. In: Neuroendocrine Tumours. Berlin, Heidelberg: Springer Berlin Heidelberg, 2015;199–221.
- Goebel SU, Heppner C, Burns AL, et al. Genotype/phenotype correlation of multiple endocrine neoplasia type 1 gene mutations in sporadic gastrinomas. J Clin Endocrinol Metab 2000;85:116-23. [Crossref] [PubMed]
- Kawamura J, Shimada Y, Komoto I, et al. Multiple endocrine neoplasia type 1 gene mutations in sporadic gastrinomas in Japan. Oncol Rep 2005;14:47-52. [PubMed]
- Chen YJ, Vortmeyer A, Zhuang Z, et al. Loss of heterozygosity of chromosome 1q in gastrinomas: occurrence and prognostic significance. Cancer Res 2003;63:817-23. [PubMed]
- Wild A, Langer P, Celik I, et al. Chromosome 22q in pancreatic endocrine tumors: identification of a homozygous deletion and potential prognostic associations of allelic deletions. Eur J Endocrinol 2002;147:507-13. [Crossref] [PubMed]
- Muscarella P, Melvin WS, Fisher WE, et al. Genetic alterations in gastrinomas and nonfunctioning pancreatic neuroendocrine tumors: an analysis of p16/MTS1 tumor suppressor gene inactivation. Cancer Res 1998;58:237-40. [PubMed]
- Serrano J, Goebel SU, Peghini PL, et al. Alterations in the p16 INK4a /CDKN2A tumor suppressor gene in gastrinomas. J Clin Endocrinol Metab 2000;85:4146-56. [Crossref] [PubMed]
- Sorbye H, Welin S, Langer SW, et al. Predictive and prognostic factors for treatment and survival in 305 patients with advanced gastrointestinal neuroendocrine carcinoma (WHO G3): the NORDIC NEC study. Ann Oncol 2013;24:152-60. [Crossref] [PubMed]
- Yachida S, Vakiani E, White CM, et al. Small cell and large cell neuroendocrine carcinomas of the pancreas are genetically similar and distinct from well-differentiated pancreatic neuroendocrine tumors. Am J Surg Pathol 2012;36:173-84. [Crossref] [PubMed]
- Tirosh A, Killian JK, Zhu YJ, et al. Oncogene panel sequencing analysis identifies candidate actionable genes in advanced well-differentiated gastroenteropancreatic neuroendocrine tumors. Endocr Pract 2019;25:580-8. [Crossref] [PubMed]
- Tang LH, Basturk O, Sue JJ, et al. A practical approach to the classification of WHO Grade 3 (G3) well-differentiated neuroendocrine tumor (WD-NET) and poorly differentiated neuroendocrine carcinoma (PD-NEC) of the pancreas. Am J Surg Pathol 2016;40:1192-202. [Crossref] [PubMed]
- Singhi AD, Klimstra DS. Well-differentiated pancreatic neuroendocrine tumours (PanNETs) and poorly differentiated pancreatic neuroendocrine carcinomas (PanNECs): concepts, issues and a practical diagnostic approach to high-grade (G3) cases. Histopathology 2018;72:168-77. [Crossref] [PubMed]
- Raymond E, Kulke MH, Qin S, et al. Efficacy and safety of Sunitinib in patients with well-differentiated pancreatic neuroendocrine tumours. Neuroendocrinology 2018;107:237-45. [Crossref] [PubMed]
- Neychev V, Steinberg SM, Cottle-Delisle C, et al. Mutation-targeted therapy with sunitinib or everolimus in patients with advanced low-grade or intermediate-grade neuroendocrine tumours of the gastrointestinal tract and pancreas with or without cytoreductive surgery: protocol for a phase II clinical trial. BMJ Open 2015;5:e008248. [Crossref] [PubMed]
- Allen A, Qin ACR, Raj N, et al. Rare BRAF mutations in pancreatic neuroendocrine tumors may predict response to RAF and MEK inhibition. PLoS One 2019;14:e0217399. [Crossref] [PubMed]
- Plass C, Pfister SM, Lindroth AM, et al. Mutations in regulators of the epigenome and their connections to global chromatin patterns in cancer. Nat Rev Genet 2013;14:765-80. [Crossref] [PubMed]
- Tirosh A, Mukherjee S, Lack J, et al. Distinct genome-wide methylation patterns in sporadic and hereditary nonfunctioning pancreatic neuroendocrine tumors. Cancer 2019;125:1247-57. [Crossref] [PubMed]
- Cejas P, Drier Y, Dreijerink KMA, et al. Enhancer signatures stratify and predict outcomes of non-functional pancreatic neuroendocrine tumors. Nat Med 2019;25:1260-5. [Crossref] [PubMed]
- Hegi ME, Diserens AC, Gorlia T, et al. MGMT gene silencing and benefit from temozolomide in glioblastoma. N Engl J Med 2005;352:997-1003. [Crossref] [PubMed]
- Lemelin A, Barritault M, Hervieu V, et al. O6-methylguanine-DNA methyltransferase (MGMT) status in neuroendocrine tumors: a randomized phase II study (MGMT-NET). Dig Liver Dis 2019;51:595-9. [Crossref] [PubMed]
- Raverot G, Sturm N, de Fraipont F, et al. Temozolomide treatment in aggressive pituitary tumors and pituitary carcinomas: a French multicenter experience. J Clin Endocrinol Metab 2010;95:4592-9. [Crossref] [PubMed]
- Ramirez RA, Beyer DT, Chauhan A, et al. The role of capecitabine/temozolomide in metastatic neuroendocrine tumors. Oncologist 2016;21:671-5. [Crossref] [PubMed]
- Li J, Woods SL, Healey S, et al. Point mutations in exon 1B of APC reveal gastric adenocarcinoma and proximal polyposis of the stomach as a familial adenomatous polyposis variant. Am J Hum Genet 2016;98:830-42. [Crossref] [PubMed]
- Mitsui Y, Yokoyama R, Fujimoto S, et al. First report of an Asian family with gastric adenocarcinoma and proximal polyposis of the stomach (GAPPS) revealed with the germline mutation of the APC exon 1B promoter region. Gastric Cancer 2018;21:1058-63. [Crossref] [PubMed]
- Ji L, Lu B, Wang Z, et al. Identification of ICAT as an APC inhibitor, revealing Wnt-dependent inhibition of APC-axin interaction. Mol Cell 2018;72:37-47.e4. [Crossref] [PubMed]
- Donninger H, Vos MD, Clark GJ. The RASSF1A tumor suppressor. J Cell Sci 2007;120:3163-72. [Crossref] [PubMed]
- Yuan Z, Claros CS, Suzuki M, et al. Loss of <i>MEN1</i> activates DNMT1 implicating DNA hypermethylation as a driver of MEN1 tumorigenesis. Oncotarget 2016;7:12633-50. [Crossref] [PubMed]
- Conemans EB, Lodewijk L, Moelans CB, et al. DNA methylation profiling in MEN1-related pancreatic neuroendocrine tumors reveals a potential epigenetic target for treatment. Eur J Endocrinol 2018;179:153-60. [Crossref] [PubMed]
- Maher ER, Iselius L, Yates JR, et al. Von Hippel-Lindau disease: a genetic study. J Med Genet 1991;28:443-7. [Crossref] [PubMed]
- Zimmer M, Doucette D, Siddiqui N, et al. Inhibition of hypoxia-inducible factor is sufficient for growth suppression of VHL-/- tumors. Mol Cancer Res 2004;2:89-95. [PubMed]
- Nielsen SM, Rhodes L, Eng C, et al. Von Hippel-Lindau disease: genetics and role of genetic counseling in a multiple neoplasia syndrome. J Clin Oncol 2016;34:2172-81. [Crossref] [PubMed]
- Lonser RR, Glenn GM, Walther M, et al. von Hippel-Lindau disease. Lancet 2003;361:2059-67. [Crossref] [PubMed]
- Keutgen XM, Hammel P, Choyke PL, et al. Evaluation and management of pancreatic lesions in patients with von Hippel–Lindau disease. Nat Rev Clin Oncol 2016;13:537-49. [Crossref] [PubMed]
- Tirosh A, Sadowski SM, Linehan WM, et al. Association of VHL genotype with pancreatic neuroendocrine tumor phenotype in patients with von Hippel-Lindau disease. JAMA Oncol 2018;4:124-6. [Crossref] [PubMed]
- Krauss T, Ferrara AM, Links TP, et al. Preventive medicine of von Hippel–Lindau disease-associated pancreatic neuroendocrine tumors. Endocr Relat Cancer 2018;25:783-93. [Crossref] [PubMed]
- Hes F, Zewald R, Peeters T, et al. Genotype-phenotype correlations in families with deletions in the von Hippel-Lindau (VHL) gene. Hum Genet 2000;106:425-31. [Crossref] [PubMed]
- Chen F, Kishida T, Yao M, et al. Germline mutations in the von Hippel-Lindau disease tumor suppressor gene: Correlations with phenotype. Hum Mutat 1995;5:66-75. [Crossref] [PubMed]
- Blansfield JA, Choyke L, Morita SY, et al. Clinical, genetic and radiographic analysis of 108 patients with von Hippel-Lindau disease (VHL) manifested by pancreatic neuroendocrine neoplasms (PNETs). Surgery 2007;142:814-8; discussion 818.e1-2.
- Krauss T, Ferrara AM, Links TP, et al. Preventive medicine of von Hippel–Lindau disease-associated pancreatic neuroendocrine tumors. Endocr Relat Cancer 2018;25:783-93. [Crossref] [PubMed]
- Tirosh A, El Lakis M, Green P, et al. In silico VHL gene mutation analysis and prognosis of pancreatic neuroendocrine tumors in von Hippel-Lindau disease. J Clin Endocrinol Metab 2018;103:1631-8. [Crossref] [PubMed]
- Neychev V, Sadowski SM, Zhu J, et al. Neuroendocrine tumor of the pancreas as a manifestation of Cowden syndrome: a case report. J Clin Endocrinol Metab 2016;101:353-8. [Crossref] [PubMed]
- Randle SC. Tuberous sclerosis complex: a review. Pediatr Ann 2017;46:e166-71. [Crossref] [PubMed]
- Mortaji P, Morris KT, Samedi V, et al. Pancreatic neuroendocrine tumor in a patient with a TSC1 variant: case report and review of the literature. Fam Cancer 2018;17:275-80. [Crossref] [PubMed]
- Asprino PF, Linck RDM, Cesar J, et al. TSC2 rare germline variants in non-tuberous sclerosis patients with neuroendocrine neoplasias. Endocr Relat Cancer 2018;25:L1-5. [Crossref] [PubMed]
- Schrader J, Henes FO, Perez D, et al. Successful mTOR inhibitor therapy for a metastastic neuroendocrine tumour in a patient with a germline TSC2 mutation. Ann Oncol 2017;28:904-5. [Crossref] [PubMed]